The global annual cancer mortality has recently reached approximately 10 million, with projections indicating a rise to 22 million by 2035 [1]. The traditional methods for clinical cancer treatment mainly include surgery, chemotherapy, and radiotherapy. Surgery can directly remove obvious solid tumors, but lacks precision in completely eradicating residual cancer cells or tissues. Moreover, some patients are physically weak and unable to undergo surgical intervention. Chemotherapy has systemic therapeutic effects, but also has systemic toxicity and significant side effects [2]. For advanced cancer, 50–70% of patients undergo radiotherapy. Radionuclide therapy uses ionized atoms and free radicals released by radionuclides to cleave single stranded DNA, thereby inducing cancer cell apoptosis [3]. In addition, radionuclides can be used for single photon emission computed tomography (SPECT) [4] and positron emission tomography (PET) [5, 6], which are the main methods for diagnosing tumors and metastases.
Radionuclide therapy faces the following challenges: certain types of cancer are insensitive to RT, non-specific distribution hinders bioavailability, and ionizing damage to non-target organs. The way to solve the above problems is to combine radionuclides with small molecule probes and nano-carriers [7,8,9]. Firstly, various biomaterials such as antibodies, peptides, liposomes, nanoparticles, have been developed for radionuclides targeted delivery [10,11,12]. By using nanoparticles to deliver radionuclides specifically to the tumor tissues, the bioavailability of radionuclides is increased and toxicity to normal tissues is reduced. By adjusting the appropriate size and surface modification nanoparticles with targeted ligand, it has been shown to prolong blood circulation time and enhance the accumulation and retention of radionuclides in tumor tissues [13]. Secondly, the tumor microenvironment (TME) is different from normal tissues, such as hypoxia, acidity, and elevated levels of reactive oxygen species (ROS) [14]. These abnormalities promote tumor invasiveness, metastasis, recurrence, and resistance to treatments including RT [15]. The nanoparticles design can regulate the TME and improve RT efficacy. Finally, high-Z element nanoparticles can be used as radiosensitizers to enhance RT, especially precious metal nanoparticles, which enhance the radiation absorption cross-section, increase the relative dose accumulation of tumors, promote free radical generation, enhance DNA damage ability, and improve RT efficacy [16].
Recently, superparamagnetic iron oxide nanoparticles (SPIONs), silica gold nanoshells, carbon nanoparticles, as well as radiopharmaceuticals labelled with radionuclides, 64Cu labelled liposomes carrying doxorubicin (Dox) and 89Zr labelled polymeric nanoparticles carrying docetaxel have been used for clinical imaging studies [17]. The first clinical PET imaging study of 64Cu labelled liposomes carrying Dox was conducted in patients with primary and metastatic breast cancer. There is a correlation between the deposition of 64Cu and Dox in tumors and patient’s disease progression free survival period [18]. In another important clinical case, 89Zr labelled polymeric nanoparticles carrying docetaxel was used in a clinical study, and it was found that the size of lesion with the highest nanoparticle uptake decreased by 50% after 96Â h of treatment [19].
In addition to imaging, the clinical therapeutic application of 188Re labelled liposomes has also been reported [20]. Therapeutic effects have been observed in some metastatic lesions, and SPECT and CT imaging also showed uptake of 188Re labelled liposomes. However, dosimetric studies have shown that the liver and spleen of patients receive the highest doses, leading to the termination of clinical work. At present, there have been no clinical studies on radionuclides labelled nanoparticles for combination therapy. However, these preliminary studies have obtained regulatory approval for clinical use [21, 22], highlighting the potential of nanoparticles in combination therapy for tumors.
Single therapy is limited by tumor resistance, and new drug development often encounters such resistance. Nanoparticles can not only achieve targeted delivery of radionuclides to tumor sites, but also be used in combination with other therapies such as PTT and CMT [23, 24]. Combination therapy involves the use of two or more methods to achieve better therapeutic effects, which is expected to reduce drug resistance, cancer metastasis, and adverse reactions. The combination of radionuclides with PTT [8], PDT [10, 25,26,27,28,29], CMT [30], immunotherapy [31], and other treatment methods to enhance the efficiency of cancer treatment.
This review briefly introduces therapeutic radionuclides, summarizes the combination therapy of RT with PTT, PDT, CMT, immunotherapy, representative research on nanoparticles labelled with radionuclides, and provides a comprehensive overview of their applications in cancer treatment. This article reviews the latest progress in the combination therapy of radionuclides based on multifunctional nanoparticles and other treatment strategies, and explores clinical studies on how to improve the combination of RT and other therapies. I hope this review can provide some guidance for the optimization of radionuclides labelled nanoparticles and corresponding treatment strategies.
Radionuclides for therapy
131I radionuclide therapy of thyroid cancer and hyperthyroidism has a history of over 70 years. Bone-targeted radionuclide therapy can be achieved using 223Ra-chloride [32], 89Sr-chloride, 153Sm-[ethylenediamine tetramethylene phosphonic acid] and 188Re-[hydroxyethylene diphosphonate] [33]. Radionuclide therapy of metastatic castration-resistant prostate cancer includes 177Lu-Prostate specific membrane antigen (PSMA) [34], 225Ac-PSMA [35], 223Ra-dichloride [36] and 188Re-Hydroxyethylidene Diphosphate [37]. The pioneer of Peptide Receptor Radionuclide Therapy (PRRT) is 111In diethylenetriaminepentaacetic acid-D-[Phe1]-Octreotide. 111In emits Auger and conversion electrons, which have potential cytotoxic effects [8]. Moreover, 90Y-DOTA-Tyr3-Octreotide [38] and 177Lu-DOTA0-Tyr3 Octreotate [39] are commonly used for PRRT.
The physical characteristics of radionuclide include decay modes, emission properties and half-life, while their biological characteristics include stability and toxicity in vivo. Moreover, significant biomedical factors include the type, size, density, and heterogeneity of targeted tumor. Figure 1 and Table 1 summarizes alpha particle (α), beta particle (β), and Auger electron (AE) decay characteristics, including half-life, emission energies, and their penetration ranges within tissues.
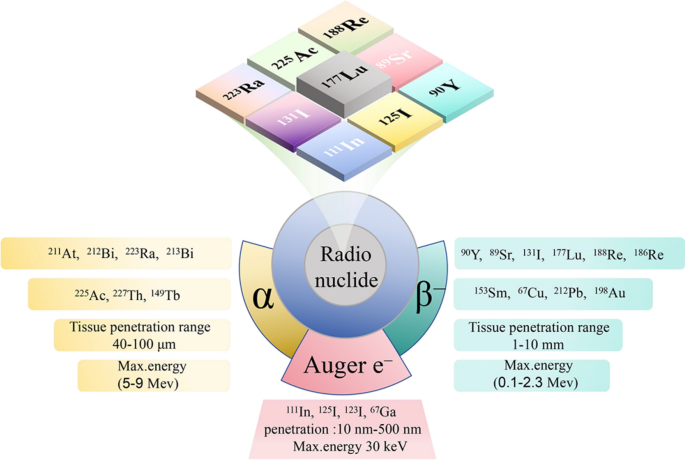
Radionuclides for radionuclide therapy [7, 8]
Alpha-particle radiation
Alpha radioactive isotopes possess lower emission energies (5–9 MeV) and smaller penetration capabilities (40–100 μm) [25]. Notably, the characteristic of α radioactive isotopes is high linear energy transfer. There was more extensive damage in specific absorption sites, increasing DNA double-strand breaks. The advantages of α-emitting nuclides stem from their higher radiobiological efficiency, being less influenced by tissue oxygenation, dose rates, and cellular resistance, while also demonstrating lower toxicity to non-target tissues [40, 41].
Beta-particle radiation
Beta radioactive isotopes possess greater emission energies (0.1–2.3 MeV) and penetration (1–10 mm), enabling the more uniform deposition of radiation dose to non-target cells by radioactive isotopes bound to adjacent target cells, even in circumstances of non-uniform distribution at the target site. However, this action may lead to radiation exposure to radiation-sensitive non-target tissues. Moreover, the cytotoxic effect of β radiation significantly relies on tumor microenvironment, particularly cell metabolism and oxygen level. It is also possible for certain tumor cells to develop tumor resistance [8].
Auger electron radiation
The energy of Auger electron is typically less than 30 keV, with a highly limited range of action, generally within 10 nm to 500 nm [42]. Auger electrons released by radionuclides typically only become effective upon entering into the cell nucleus. Metabolites carrying Auger electrons selectively and locally irradiate the cell membrane and DNA, inducing cell death [7].
Photothermal therapy
Photothermal therapy represents a non-invasive therapeutic approach, employing near-infrared light (NIR) to irradiate tumor areas with minimal impact on normal tissue [43]. Tumor cells exhibit weaker tolerance to high temperatures compared to normal cells, and temperatures of around 45 °C can induce apoptosis [44, 45]. Combination therapies of radionuclides and photothermal therapy include polydopamine (PDA), Pd nanosheets, Au nanoparticles. Except PTT, Magnetic hyperthermia (MHT) with magnetic iron oxide nanoparticles also exhibits notable therapeutic efficacy (Table 2).
Polydopamine
Polydopamine has inherent biocompatibility, simple preparation, strong near-infrared absorption, high photothermal conversion efficiency, and easy surface modification. As a multifunctional material, PDA has been widely used in biomedical fields [46]. Liu et al. [47] encapsulated single-walled carbon nanotubes with a polydopamine shell, modified with poly(ethyleneglycol) (SWNT@PDA-PEG). SWNTs exhibit strong NIR absorbance. 131I was labelled to SWNT@PDA-PEG for imaging and radionuclide therapy. Both magnetic resonance imaging (MRI) and gamma imaging demonstrated effective SWNT@PDA-131I-PEG tumor accumulation. Compared to monotherapies, the combined approach of NIR-triggered PTT and 131I radionuclide therapy resulted in tumor eradication with a remarkable synergistic anti-tumor effect. Anaplastic thyroid cancer is one of the most aggressive malignancies in humans, and tumor cells are resistant to 131I uptake. Wang et al. [48] synthesized mesoporous PDA nanoparticles with a brain-like channel structure. PDA not only serve as 131I nanocarrier with large specific surface area, but also function as photothermal conversion agents for PTT. PDA + NIR rapidly heated the tumor tissue within 6 h post-injection, reaching 50 °C within 5 min, far surpassing control group. Impressively, as a result of the combination of PTT and RT, animal models treated with PDA-131I NPs exhibited an impressive therapeutic response. Li and co-workers [49] developed PDA and surface-functionalized gold nanoparticles (PDA-131I-AuNFs-PEG). SPECT confirmed that 131I-AuNFs exhibited high photothermal conversion efficiency, stable radioactivity and effectively accumulating in vivo. Under 1064 nm lasers, PTT combined with RT, the treatment outcome far surpassed that of either single therapy. Injection of AuNFs and PDA-131I-AuNFs-PEG into tumor-bearing mice resulted in 57.8% and 100% suppression of tumor growth, respectively.
In addition to PDA, researchers have also studied other organic nanoparticles labelled with radionuclides. Meng [50] synthesized 131I-labelled Human serum albumin-Indocyaninegreen (131I-HSA-ICG) nanoparticles ranging in size from 25 to 45 nm. These nanoparticles exhibited a photothermal conversion efficiency of up to 24.25% under NIR laser. Fluorescent imaging and SPECT confirmed that 131I-HSA-ICG nanoparticles could persist in tumor tissues for 4–6 days. Under 808 nm laser, 131I-HSA-ICG demonstrated significant ablation on tumor. Zhu and co-workers [51] developed a novel biocompatible melanin-based nanoprobe (PMNs-II-813) coupled with highly specific small-molecule inhibitors of prostate-specific membrane antigen, designed for precise multimodal diagnosis and treatment of prostate cancer. The Melanin exhibited photoacoustic imaging and photothermal therapy capabilities under NIR laser. 89Zr, 131I and Mn2+ were stably conjugated to the nanoparticles, guiding RT and PTT under PET/MRI multimodal imaging. The combination of PTT and RT suppressed prostate cancer growth (approximately 93% tumor suppression rate 20 days post-treatment), significantly outperforming single therapy.
Au
Au nanoparticles possess remarkable qualities like high inertness, excellent biocompatibility, ease of chemical modification, and efficient transfer capabilities. It has garnered significant attention and undergone extensive research in the domain of photothermal therapy [13]. A variety of Au nanostructures with diverse sizes and shapes, spherical nanoparticles, cubic nanostructures, nanorods, and hollow nanoshells, have been precisely synthesized under controlled conditions. Jeon [52] developed 124I labelled gold nanoparticles (124I-Au) as a therapeutic nanoplatform. 124I-Au exhibited effective photothermal conversion both in vitro and vivo, efficiently absorbed by macrophages without cytotoxicity. Macrophages with 124I-Au were administered to colon tumors, revealing strong signals at the tumor site. Following NIR laser excitation, the temperature increased to above 43 °C, resulting in a significant tumor reduction in mice treated with 124I-Au + NIR laser. Combination therapy can significantly enhance therapeutic efficacy against nonspecific targeting of anticancer drugs. Zyuzin et al. [53] utilized a nanocarrier (gold nanorods and paclitaxel) labelled with therapeutic radionuclide 188Re (Fig. 2). PTT combined with RT and chemotherapy exhibited significantly improved efficiency compared to monotherapy. Peptide Receptor Radionuclide Therapy (PRRT) relies on the binding of peptides with α and β-emitting radionuclides, requiring high doses which may lead to potential toxicity. Kjaer [54] investigated 177Lu-DOTA-TATE along with gold nanoshells for PTT in treating small cell lung tumors expressing somatostatin receptors. PTT, relying on the heat induced by gold nanoparticles, complemented PRRT. Compared to PRRT alone, mice treated with gold nanoshells PTT combination therapy 1 day after injection of 177Lu-DOTA-TATE showed suppressed tumor growth and longer survival time.
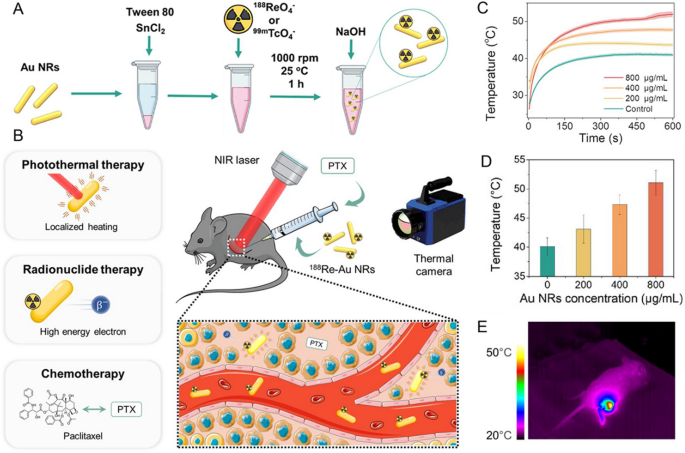
Source: Development of Nanocarrier-Based Radionuclide and Photothermal Therapy in Combination with Chemotherapy in Melanoma Cancer Treatment
A, B Combination therapy using 188Re/99mTc-labelled Au NRs and Paclitaxel (PTX). C Temperature evolution curves of Au NRs at different concentrations. D Average temperature reached after 600 s of NIR laser irradiation of tumors with injected Au NRs at varying concentrations. E Thermal images of a mouse with intratumorally injected 800 μg/mL Au NRs irradiated with 1064 nm NIR laser. Copyright {2023} American Chemical Society.
Pd nanosheets
Pd nanosheets, as a photothermal agent, has good biocompatibility, high photothermal conversion efficiency, and urine clearance rate. Through structural engineering, such as ultra-thin Pd nanosheets, their photothermal effect can be enhanced, and NIR absorption can be improved [55]. Zheng and co-workers [56] have reported a pH-sensitive multifunctional therapeutic platform, 131I and 125I labelled Pd nanosheets. 131I-Pd-PEG is relatively stable in acidic or slightly acidic solutions but unstable in neutral and slightly alkaline solutions, making it an ideal tumor microenvironment-sensitive therapeutic nanoplatform. Improved therapeutic effects were observed in mice with suppressing tumor growth upon injection of 131I-Pd-PEG. In another study conducted by the same group [57], fluorinated palladium nanosheet labelled with various radionuclides was used for guiding combination therapy. Fluorinated Diethylenetriaminepentaacetic dianhydride-PEG-SH (FDP-Pds) was labelled with 124I, 125I, 131I, 177Lu, 99mTc, and 64Cu. Under laser excitation, the temperature at the tumor site rapidly increased from 37 °C to 50 °C in 7 min, demonstrating excellent photothermal therapy. 125I/131I-labelled probes for RT exhibited outstanding therapeutic effects. The therapeutic effects of 131I-FDP-Pds + laser group markedly surpassed those of the remaining groups, with no observed recurrence during a 2-month follow-up period.
Superparamagnetic iron oxide nanoparticles
Magnetic hyperthermia uses magnetic nanoparticles to generate heat under an external alternating magnetic field, uniformly heating tumors, and has been studied to overcome RT resistance [58]. Stanković [59] conducted research on 131I-CC49 antibodies, which were conjugated to superparamagnetic iron oxide nanoparticles functionalized with 3-aminopropyltriethoxysilane (APTES). This conjugation exhibited exceptional heating efficiency, with a specific absorption rate of 15.9 kA∙m−1. After application to LS174T human colon carcinoma xenografts, it provided specific and sustained local retention. The combination of magnetic hyperthermia and RT resulted in tumor volume suppression rates of 73.00%, significantly inhibiting tumor growth. Bilewicz [60] reported a core–shell nanoparticle (SPIONs coated with a layer of 198Au). This nanoparticle exhibited a high saturation magnetization, capable of reaching 43 °C at a magnetic field frequency of 386 kHz. The vitro experiment results indicated a significantly cytotoxic effect. At a radioactive concentration of 2.5 MBq/mL, cell survival rates had dropped to below 8% after 72 h. Moreover, this group [61] employed 225Ac-modified magnetite nanoparticles, which has no compromise to its magnetic properties. The 225Ac@Fe3O4-3-phosphonopropanoic acid-trastuzumab bioconjugate, covalently linked through phosphonopropanoic acid, specifically targeted ovarian and breast cancer cells. This bioconjugate displayed high cytotoxicity against SKOV-3 ovarian cancer cells. Its high specific uptake enables it to achieve better therapeutic results in the combination of RT and magnetic hyperthermia. Combining therapies represents a promising strategy in cancer treatment. PEI-Mn0.5Zn0.5Fe2O4 nanoparticles (PEI-MZF-NPs) functioned as both the magnetic medium for magnetic fluid hyperthermia and as gene transfer vectors for gene therapy. Lin [62] developed PEI-MZF-NPs with anti-afpmcab monoclonal antibody for targeting. The suspension exposed to a magnetic field rapidly increased in temperature, maintained at around 43 °C, significantly inhibiting the proliferation of liver tumor cells, far surpassing chemotherapy with doxorubicin. The combination therapy of RT and magnetic hyperthermia demonstrated significantly superior therapeutic effects compared to single therapy.
Photodynamic therapy
Photodynamic therapy stands as a non-invasive therapeutic approach, offering a comparably lower incidence of side effects than traditional treatments. Under photoexcitation, the photosensitizer (PS) transitions from the ground state to excited state and then to longer-lived, lower-energy triplet excited state. In Type I PDT, the photosensitizer reacts with biological substrates to form ROS, such as hydroxyl radicals, hydrogen peroxide, and superoxide anions. In Type II PDT, the triplet excited state of the photosensitizer directly transfers energy to O2, converting it to singlet oxygen (1O2) [63]. The lifetime of ROS ranges from 0.03 to 0.18Â ms, thus exerting toxicity only to cells in close proximity to the photosensitizer. There is an urgent need for a new strategy to address the limitations of conventional nanoparticle-based PDT, such as tissue penetration constraints, reliance on external light sources, and limited accumulation of photosensitizers.
Photosensitizers excited by Cherenkov radiation (CR) can break the limitations of external light penetration. Discovered in 1934 by Soviet physicist Pavel Alekseevich Cerenkov [64], Cherenkov radiation occur local polarization along their trajectory, when charged particles moving faster than the speed of light in a medium. The medium releases stored energy as visible photons, which results in the characteristic blue light of Cherenkov radiation. The radionuclides can induce photosensitizers to generate ROS by Cherenkov radiation energy transfer [65, 66]. The intensity of radiation is directly related to the particle’s energy, enabling precise administration of therapeutic radiation doses to the tumor site. Moreover, the detection of CR can be utilized for real-time monitoring of PDT, contributing to the optimization of subsequent treatment plans. These benefits underscore the potential of CR-PDT as a secure and efficient approach to cancer therapy (refer to Table 3).
Porphyrin
Porphyrin and its derivatives are commonly used for PDT, and demonstrate inherent tumor-targeting properties, which positions porphyrin derivatives paired with suitable therapeutic radionuclides as potential candidates for targeted therapy. Deng [67] developed 188Re-labelled tetra-phenyl porphyrin (TPPS4). Similarly, Luo et al. [68] prepared 188Re-labelled tetra-[3,4-bis(carboxymethyleneoxy)phenyl] porphyrin. Both research groups studied characteristics such as yield, specific activity, in vitro stability, affinity, and biodistribution. 188Re-TPPS4 exhibited high tumor affinity and sustained accumulation characteristics in tumors, but its therapeutic efficacy was not evaluated. Venakatesh [69] synthesized a water-soluble 177Lu-5,10,15,20-tetra[4-carboxymethoxyphenyl]porphyrin, with a specific activity of 550 TBq/g and a radioactive isotope purity of 99.98%. It displayed 99% radiochemical purity and stability. In a preliminary efficacy study on a mouse model fibrosarcoma tumor, tumor growth was significantly suppressed. As for targeted PDT, Watanabe [70] designed a simple in vivo pharmacokinetic evaluation system. The gastrin-releasing peptide receptor was used as tumor-targeting peptide. Conjugate of Protoporphyrin IX (PPIX) and the bombesin (BBN) was synthesized as targeted agent with 64Cu labeling. [64Cu]PPIX-PEG6-BBN exhibited high uptake in PC-3 cells. Due to PPIX’s lipophilicity, it rapidly accumulated in the liver and kidneys, had a long circulation time in the blood and lower distribution in the tumor. Khalaj [71] prepared 166Ho-labelled 5,10,15,20-tetra(3,4-dimethoxyphenyl) porphyrin (TDMPP) and 5,10,15,20-tetra(3,4,5-trimethoxyphenyl)porphyrin (TTMPP), both exhibiting favorable radiochemical purity and specific activity. These two compounds demonstrated less hepatic accumulation, thus warranting attention for their potential therapeutic properties. Luo et al. [72] prepared 131I-labelled 5,10,15,20-tetrakis (4-hydroxyphenyl) porphyrin (TPPOH) and 5,10,15,20-tetrakis (4-aminophenyl) porphyrin (TPPNH2) for both RT and PDT. Upon 400–600 nm photoexcitation, the tumors treated with 131I-TPPOH and 131I-TPPNH2 exhibited a reduction in tumor volume of 64 ± 11% and 54 ± 10%, respectively, 14 days post-treatment.
Cherry et al. [73] utilized Y-90 as Cherenkov radiation to induce porphyrin-based photosensitizer tetrasulfonated alkylated metalloporphyrin (TPPS2a). TPPS2a exerted at least 20% therapeutic efficacy against C6 glioma cells within the range of 6–60 µCi/well. The current targeting efficiency of radionuclide-labelled nanoparticles is quite low, thereby restricting the efficiency of CR-PDT. Cai et al. [74] developed 89Zr-labelled deferoxamine-porphyrin nanocomplex (89Zr-Df-PPN). High doses of Df-PPN were used as CR energy receivers for passive tumor targeting, while low doses of 89Zr-labelled Df-PPN acted as CR energy donors. Based on homology, Df-PPN and 89Zr-Df-PPN were co-localized to the maximum extent at the tumor site, CR-PDT strategy achieving considerable damage to tumor blood vessels and inhibited tumor growth significantly. Optimized temporal and spatial settings maximized the efficacy of targeted CR-PDT and simultaneously reduced off-target effects. This group [75] also investigated a size-controllable dual-tumor-mitochondria-targeting porphyrin-PEG (PPN). Effective uptake of 177Lu-PPN by tumors and mitochondria significantly enhanced the efficacy of RT and PDT. Tumor growth was completely suppressed after 14 days with 177Lu-PPNs-10 nm under laser 660 nm. In another study, this group [76] synthesized magnetic nanoparticles (MNPs) with surface modification with 89Zr and porphyrin (89Zr-MNPs/TCPP) for CR-PDT and magnetic targeting tumor. Tumor accumulation enhanced by external magnetic fields. The tumor growth of mice treated with 89Zr-MNPs/TCPP was significantly suppressed.
The strategy of employing combination therapy involving two or more therapies aims to overcome tumor resistance experienced with single therapies. Cai et al. [77] assembled copper sulfide nanoparticles on the exterior of 89Zr-labelled hollow mesoporous silica nanoshells filled with porphyrin molecules, aiming to imaging and therapy. The synergistic interaction between copper-mediated PTT and porphyrin-mediated PDT resulted in complete tumor eradication within a day, with no apparent relapse or side effects. Furthermore, this approach achieves simultaneous quadmodal (Positron emission tomography/Cerenkov luminescence/Cerenkov radiation energy transfer/Fluorescence) imaging, enabling the application of multimodal image-guided therapy. Qiu et al. [78] combined tetraphenylporphyrin (a photosensitizer) and hapten (a chemotherapeutic drug) using cleavable sulfone linkers to produce a ROS-responsive agent. Under 68Ga Cerenkov radiation excitation, porphyrin generated ROS, which subsequently cleaved the sulfur-based linkers, leading to internal agent release. 68Ga-NOTA-Nb109 as an intracellular CR emitter, this ROS-responsive agent exhibited significantly higher toxicity to A375-hPD-L1 cells, owing to the combination of PDT and CMT.
Titanium dioxide
Titanium dioxide (TiO2) nanoparticles are considered to be biocompatible and cost-effective therapeutic functional nanomaterials. TiO2 nanoparticles have been proven effective as photosensitizers for cancer treatment. The bandgap energy of TiO2 is 3.2 eV. Under CR excitation, the ground state of TiO2 nanoparticles transitions to excited state and generates ROS. Hilefu [79] reported that 18F-FDG induced an O2-independent photosensitizer, transferrin-coated TiO2 nanoparticles (TiO2–Tf). Within three days, tumor volume notably decreased by 40 ± 5%. Non-invasively tracking the distribution of TiO2 NPs in the body remains elusive. This group [80] loaded Gd onto transferrin-coated TiO2 NPs. TiO2-Gd-Tf maintained ROS generation capability at wide concentrations and induced significant cell death. Gd contrast agents facilitated MRI monitoring of NPs distribution and accumulation within tumors. Liu et al. [81] compared 68Ga and 18F, demonstrating that 68Ga is a more effective CR-PDT radionuclide. The Cherenkov efficiency of 18F is relatively low, requiring dosages 10–30 times higher than usual to achieve therapeutic effects. 68Ga as CR source to induce D-glucose-modified TiO2, 68Ga-labelled bovine serum albumin (68Ga-BSA) exhibited suppression of 4T1 cell growth compared to 18F labelled 2-fluoro-deoxyglucose (18F-FDG). Achilefu [82] developed a therapeutic nanocomposite, TiO2 coated with tumor-targeting transferrin and 89Zr (Fig. 3A). 89Zr-TiO2-Tf generated ROS, triggering apoptosis and ultimately reducing the tumor volume by tenfold. AcPark [83]developed an CR-induced 89Zr-TiO2-MnO2 nanocomposite, which binds transferrin to the nanoparticle surface, inhibiting cancer cell growth. MnO2 converted tumor area acidic H2O2 into O2, thereby enhancing the efficacy of CR-PDT. Overexpressed glutathione in tumor cells can be depleted by MnO2, disrupting the antioxidant defense system and promoting PDT (Fig. 3B, C). Moreover, inherent MnO2 can absorb Cherenkov radiation, facilitating ROS generation and inhibiting tumor growth. Different system parameters (nanoparticle size, nanoparticle concentration, and radioactivity of radionuclides) can influence the quantity of Cherenkov photons and ROS generation. Biswas [84] developed a mathematical model that integrates Cherenkov physics, light-matter interactions and photocatalytic reaction. This model described the pathway of CR-induced 18F-FDG/TiO2 to generate ROS. The generated ROS increased with the increase of TiO2 concentration. The concentration of ROS first increased with the increase of particle size, reached a peak at around 800 nm, and then decreased. This was mainly due to the dominance of photoexcitation and e−/h+ generation when the particle size is below 800 nm.
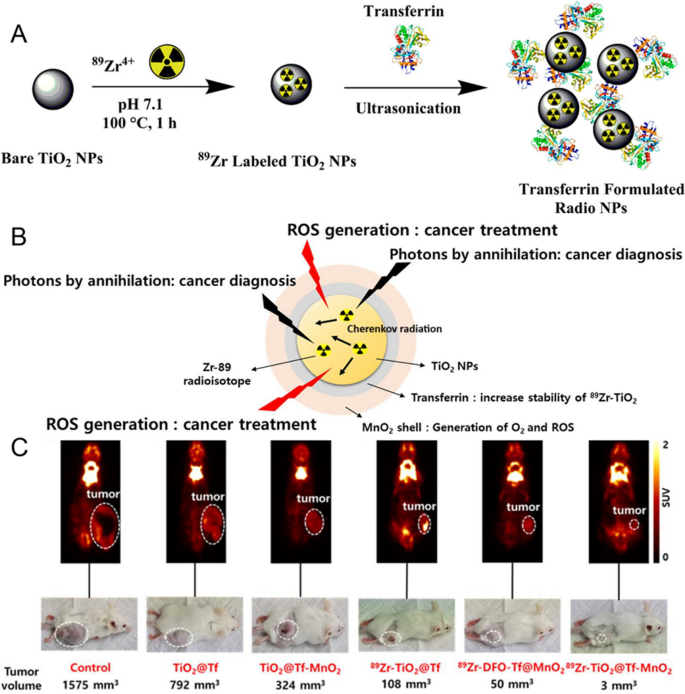
Source: Osteotropic Radiolabeled Nanophotosensitizer for Imaging and Treating Multiple Myelom, Theranostics through Utilizing Cherenkov Radiation of Radioisotope Zr-89 with a Nanocomposite Combination of TiO2 and MnO2
A Schematic illustration of 89Zr-labelled of TiO2 NP and 89Zr-TiO2-Tf. Copyright {2020} American Chemical Society. B Type of theranostic 89Zr − TiO2@Tf-MnO2. C.18F-FDG PET images and tumor photographs after CR-PDT. Copyright {2023} American Chemical Society.
Other photosensitizers
The research on photosensitizers for PDT mainly focuses on organic nanoparticle porphyrins and inorganic nanoparticle TiO2. Other types of photosensitizers have also been studied in combination therapy. Gao et al. [85] developed 131I-labelled 5-aminolevulinic acid onto exosome-mimetic vesicles (131I-EM@ALA). Besides radionuclide therapy, 131I was also used to CR light source. Through the combination of RT and CR-PDT, 131I-EM@ALA induced DNA damage and activated the lysosomal-mitochondrial pathway to kill tumor cells. In comparison to the control group (0%, 24 days), the 131I-EM@ALA group showed significantly prolonged survival rates (12.5%, 35 days). In the context of disseminated multiple myeloma cells, Achilefu et al. [86] reported a high-selective delivery and co-localization nanocomposite composed of HSA, transferrin, titanocene and 18F-FDG. The CR-induced nanocomposite demonstrated suppression of tumor growth in highly metastatic breast cancer models. Downregulation of dimeric protein targets CD49d resulted in enhanced therapeutic resistance in small cluster carcinoma cells, thereby expanding the application to previously untreatable metastatic diseases.
Injecting radionuclides and photosensitizers separately don’t guarantee their effective interaction in the tumor site. A common approach to address this issue is through the nanoparticles self-assembly. Sun [87] developed a self-assembly 131I-labelled photosensitizer (131I-sPS), which composed of porphyrin-a (photosensitizer), diisopropyl (pH-sensitive moiety), and 131I-labelled tyrosine (CR supplier). Due to aggregation-induced quenching, 131I-sPS exhibited lower photo-toxicity in normal tissues, but could generate ROS at the tumor site upon decomposition. Following intravenous injection, it demonstrated excellent tumor inhibition in subcutaneous 4T1-tumor mice. Miao [88] developed a glycol chitosan-based nanoparticles (GCP-NPs) consisting of pyropheophorbide-a. GCP-NPs exhibited pH-dependent surface charge conversion, and switched its surface charge from negative to positive in acidic tumor microenvironment, enhancing cellular internalization and penetration, improving the tumor accumulation. Moreover, 99mTc of SPECT imaging in a subcutaneous mouse tumor model showed an 8.1-fold increase in tumor uptake compared to GCP-NPs. Compared with other groups, 177Lu-GCP-NPs combined RT with PDT, demonstrated an ideal tumor growth suppression rate of 95.5% (Fig. 4).
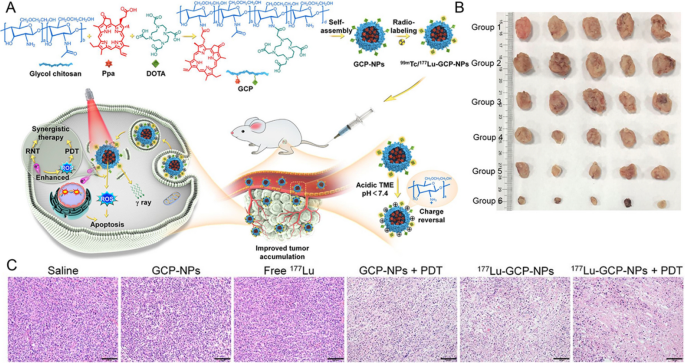
Source: Acidity-Activated Charge Conversion of 177Lu-Labeled Nanoagent for the Enhanced Photodynamic Radionuclide Therapy of Cancer
A Schematic illustration of 99mTc/177Lu-GCP-NPs and the mechanism of combination therapy. B Photographs of tumors after treatments. C Histological H&E staining of tumors after treatments. Scale bar: 100 μm. Copyright {2022} American Chemical Society.
Cai et al. [89]synthesized hollow mesoporous silica nanoparticles (HMSN) loading Ce6 and 89Zr. Ce6 could be induced by 89Zr CR. Vivo studies revealed that subcutaneous injection of 89ZrHMSN-Ce6 had a suppressive effect on tumor growth, resulting in a 75% reduction in tumor volume. Zhang [90] developed ultra-small nanoclusters, derived from NH2-Ti32O16. The introduction of dopamine (DA) ligands not only promoted the water solubility and photocatalytic performance, but also engaged in tumor-targeting. Under 18F-FDG CR excitation, it generated hydroxyl radicals, where holes (h+) reacted with H2O to perform type I PDT, and the transferred e− achieved Ti3+ enhancement, significantly improving chemotherapy (Fig. 5). Radionuclides and CR can be used as internal light sources to induce photosensitizers for PDT of deep tumors. However, the low efficiency of CR limits its therapeutic effect. Sun et al. [91] developed a 131I-labelled zinc tetra(4-carboxyphenoxy) phthalocyaninate, which conjugated Cr3+ doped zinc gallate nanoplatform (131I-ZGCs-ZnPcC4) for RT and CR-PDT. 131I could induce ZGCs by Cherenkov luminescence and ionizing radiation, allowing it to sustainably emit light and induce ZnPcC4 photosensitizer for PDT. 131I-ZGCs-ZnPcC4 demonstrated excellent tumor suppression both in vitro and vivo. In combination therapy of RT and PDT, PDT is mostly induced by CR. Including radionuclides 131I, 89Zr, 18F, etc. CR radiation energy is low, and some researchers have introduced high-energy external light sources. Rijpkema [92] synthesized high-affinity and highly tumor-targeting 111In-labelled nanocomposite composed of PSMA ligands, fluorophore, and IRDye700DX. Compared to white light, nanocomposite under 690 nm excitation for fluorescence-guided surgery resulted in reduced tumor recurrence and significantly prolonged survival.
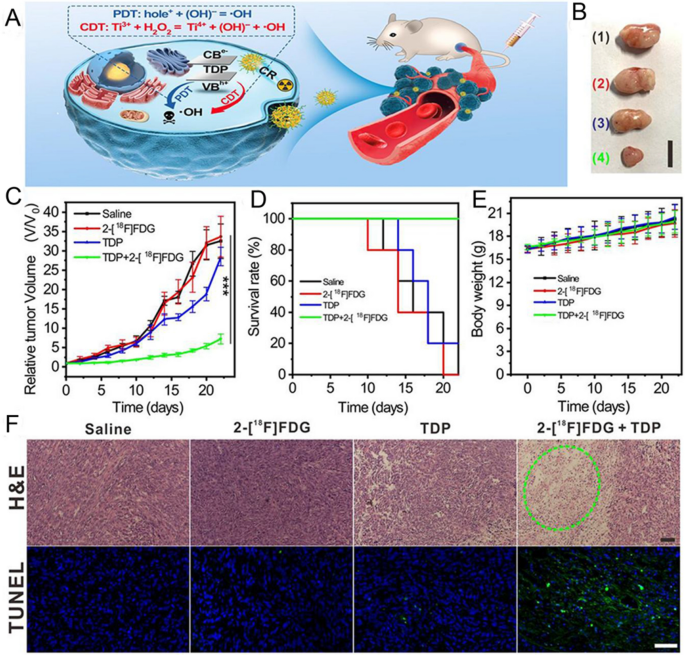
Source: Ligand Engineering of Titanium-Oxo Nanoclusters for Cerenkov Radiation-Reinforced Photo/Chemodynamic Tumor Therapy
A Schematic illustration of combination therapy. B Size of tumor at the 22nd day post treatment in each group from up to below: saline, 2-[18F] FDG, TDP, and TDP + 2-[18F] FDG (scale bar: 1 cm). C Average tumor growth curves after treatments. D Survival curves after treatments. E Weight growth curves after treatments. F Representative images of the tumor dissection stained by H&E and TUNEL after treatments. (Scale bar: 50 μm). Copyright {2021} American Chemical Society.
Chemotherapy
The concept of combination therapy originates from chemotherapy, integrating the localized or systemic administration of various radiolabeled-molecules, chemotherapeutic agents, or other compounds. This combination of RT and CMT offers a more personalized approach to cancer treatment. Nanoscale platform, such as liposome and micelle, allows for the encapsulation of multiple therapeutic compounds and radionuclides. Moreover, targeting modification with antibodies, antibodies fragments, and peptides facilitate the amalgamation of various therapies. Chemotherapeutic agents studied in combination with RT include doxorubicin, paclitaxel and alkylating-agents et al. (Table 4).
Doxorubicin
Doxorubicin is a widely used anticancer anthracycline antibiotic that accumulates in the nucleus. Shieh [93] loaded Dox onto multifunctional micelles. Tumors treated with 188Re-Dox micelles reduced cellular proliferation and exhibited more necrotic features. Liu [94] discovered 131I-99mTc-PDA-PEG/Dox nanoparticles for the combination of tumor targeted radiotherapy and chemotherapy. The murine tumor model exhibited significant efficiency in cancer treatment compared to single therapy. Moreover, no significant toxicity was observed in treated mice within 80 days. Ningthoujam [95] developed 177Lu-labelled NaGdF4/Ho-Yb up-conversion nanoparticles, coated with a biocompatible m-SiO2 layer and Dox. Under 980 nm excitation, up-conversion nanoparticles emit red and green light. Following the action of NaGdF4/Ho-Yb@mSiO2-Dox, enhanced elimination of cancer cells was observed. Pilip [96] synthesized 213Bi-labelled cubic liquid crystalline phases with the Dox and amphiphilic ligand, which resulted in a significant decrease in survival capability of HeLa cancer cells.
Auger electrons are very low-energy electrons emitted by radionuclides. This energy is deposited within a very short range (< 0.5 µm), resulting in high linear energy transfer and lethal damage to cancer cells. Therefore, AEs have great potential in cancer treatment. Ogawa [97] developed a 125I-labelled Dox liposome for AEs therapy. The liposome accumulated highly in cancer tissue, upon heat induction, released 125I-labelled Dox derivative into the target site. 125I-Dox derivative accumulated in nucleus, resulting in high cancer cell toxicity by emitting AEs. Thisgaard [98] prepared AEs-emitting 125I-5-iodo-2-deoxyuridine (125I-UdR) in combination with thymidine synthase inhibitors, methotrexate, and temozolomide. The combination therapy significantly decreased the viability of glioblastoma cells in contrast to 125I-UdR alone.
Paclitaxel and camptothecin
Paclitaxel (PTX) has been approved by the Food and Drug Administration as a radiation sensitizing drug. Chilkoti [99] investigated the synergistic effects of 131I-labelled thermoresponsive elastin-like polypeptide and PTX nanoparticles. Nanoparticles conjugated with albumin and paclitaxel, successfully surmounted drug resistance, demonstrating therapeutic efficiency against tumors in both subcutaneous and in situ pancreatic tumor models. Denkova [100] reported polymer micelles with PTX and 177Lu, combining CMT with RT, demonstrating better cell growth inhibition and cytotoxic effects. Xi [101] developed a 131I-labelled hybrid hydrogel nanoparticle, self-assembled with carboxymethyl cellulose (CMC), bovine serum albumin, and camptothecin for combination therapy. It exhibited a substantial drug-loading capacity, a low hemolysis rate, and pH-dependent drug release, significantly reducing damage to normal tissues. Moreover, it effectively promoted intracellular uptake, prolonged blood circulation time, and enhanced accumulation within tumor tissues. The combination therapy had shown excellent in vivo anti-cancer effects, significantly better than monotherapy.
Immunotherapy
Individual RT has limited penetration depth and uneven dose distribution, resulting in a “blind spot” of residual live cancer cells and increasing the risk of tumor metastasis. RT can stimulate anti-tumor immune response by inducing immunogenic cell death [102, 103]. The integration of radionuclide therapy with immunotherapy (internal radio-immunity therapy, IRIT) aims to accomplish effective synergism (Table 5). Yang and co-workers [104] developed a 131I-labelled delivery platform based on bacterial outer membrane vesicles, coating with tyrosine-rich protein statherin and PEG. It could stimulate dendritic cell maturation and generate anti-tumor cytokines, strengthen the systemic immune memory effects induced by radioimmunotherapy and inhibit tumor invasion.
Near-infrared photoimmunotherapy (NIR-PIT) is an approach that binds to antibody specificity but is limited by low tissue penetration of NIR light. Kobayashi [105] reported 18F-FDG CR-induce IR700 for PIT. While CR-PIT can induce deep tumor destruction in vivo, its efficiency is not as pronounced as that of NIR-PIT due to the shorter-wavelength light. Moreover, the efficacy of both RT and immunotherapy is hindered by the tumor microenvironment and immunosuppression. Liu et al. [106] developed ZnFe(CN)5NO monolayer nanosheets and proposed the use of in-situ chemiluminescence to release NOÂ for tumor microenvironment modulation. Upon 32P labeling, CR induced ZnNO (32P) nanosheets to release NO, which not only inhibited HIF-1a expression, alleviated tumor hypoxia, and enhanced the efficacy of RT, but also regulated the immunosuppressive tumor microenvironment. Combining anti-programmed cell death protein 1 with ZnNO (32P) nanosheets could activate distant effects in immune checkpoint blockade therapy, inhibiting the growth of distant metastatic tumors.
Bu and co-workers [107] reported 131I-labelled Au nanoparticles and double-arginine translocation peptide (131I-AuNPs-TAT). 131I provided high concentration of beta radiation to the most radiation-sensitive DNA. Simultaneously, when the velocity of beta particles decreased, electrons rushed to the AuNPs, leading to the conversion of low-dose X-rays. X-rays have a greater depth of penetration than beta-rays and can induce a strong immune reaction, thereby reducing the unseen areas by short-range beta radiation. Combining RT with immunotherapy not only enhances the efficacy of RT but also broadens the 131I clinical applications. Compared to control group, the tumor growth of was significantly suppressed in the 131I-AuNPs group and the 131I-AuNPs-TAT group. Yang [108] synthesized 99mTc/177Lu-labelled glutathione-modified Au nanoclusters. RT induced immunogenic cell death, activating immune cells, regulating the expression of tumor cell PD-L1. This indicated that anti-PD-L1 blockade has significant synergistic effects in inhibiting distant tumors, providing long-term immune memory protection, and significantly improving the quality of life and lifespan of transgenic mice. Despite Au nanoparticles being an inert metal that should not disrupt normal physiological functions, studies [109] have found that Au nanoparticles can stimulate immune responses and impact treatment results. AuNPs have been shown to alter gene expression associated with detoxification, lipid metabolism, and hepatic defense responses. Larger AuNPs (50 nm) rapidly increased the expression of IL-1β, IL-6, and TNF-α in organs. However, smaller AuNPs (10 nm) only led to a milder hepatic cytokine response.
Chemodynamic therapy (CDT) utilizes Fenton or Fenton-like reactions to regulate the immunogenic tumor microenvironment. However, relying solely on CDT is not adequate to activate immune responses for suppressing tumor metastasis and recurrence, a necessity arises for combination therapies of immunotherapy and RT. Yu et al. [110] designed a 211At-labelled Mn-based radioimmunotherapy promoter. 211At was used to targeted alpha therapy. Besides serving as a carrier, MnO2 generated •OH through CDT and enhanced immune checkpoint blockade (ICB), inhibiting the primary tumor without recurrence.